Scientists break down lignin to enter a world of sugar and energy

Sugar and energy locked up
Lignin is the cementing agent that holds plant cell walls together. For bioenergy researchers, lignin and other cell-wall components are significant stumbling blocks to unlocking the enormous energy that’s tied up in plants. Because once you can crack the hard lignin-boundary, you enter a world of sugars - the building blocks of the carbohydrate economy and of a huge quantity of biofuels.
Scientists are now trying to develop so-called 'third generation' biofuels, that are marked by two main features: (1) they make use of efficient biochemical and thermochemical conversion processes aimed at breaking down the lignin and hemicellulose in order to free the sugars contained in the biomass, but (2) critically, the energy crops themselves have been engineered in such a way that the plant's cells contain less lignin (image, click to enlarge). For them to succeed, they need to understand better how plants make lignin.
Plants use three main materials to build their cell walls: the polysaccharides cellulose and hemicellulose and the phenolic polymer lignin. Cellulose is a chain of glucose (sugar) molecules strung together. As these molecules multiply, they organize themselves in linear bundles that crisscross through the cell wall, giving the plant strength and structure.
The cellulose bundles are weakly bound to an encircling matrix of hemicellulose, which is strongly linked to lignin. The gluey lignin polymer further strengthens plants and gives them flexibility. Lignin is the reason plants can pop back up after heavy rains and winds. And it’s how they made the leap from a life in the ocean to one on land eons ago.
Plants have invested great energy in crafting exquisite cell-wall structures that resist degradation and loss of their precious sugars. Over the course of millions of years, they’ve had to fend off an insatiable crowd of energy-hungry fungi, bacteria, herbivores—and now, people.
A tricky and sticky plasticity
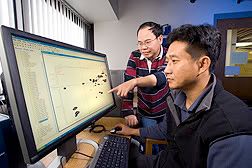
Almost 10 years ago, Ralph and colleagues published a paper describing what happens to loblolly pine trees when they’re deprived of the gene that codes for cinnamyl alcohol dehydrogenase—an enzyme that helps make vital lignin building blocks. Ralph says that even with extremely low levels of the important lignin-building enzyme, the trees compensated by incorporating novel monomers—small molecules that can bind with others to form polymers—to ensure that they had the necessary lignin-like glue to perform basic functions:

After having used NMR and other methods to analyze many other genetically transformed plants—including tobacco, aspen, alfalfa, corn, and the model plant Arabidopsis—Ralph and his colleagues and collaborators have laid a foundation of basic knowledge about how lignin production is orchestrated in plants.
Two lignin camps
Ralph belongs to a major camp of scientists who maintain that the formation of the lignin polymer is pretty much a random affair—not strictly controlled by proteins and enzymes like many other plant polymers are. Another group declares that lignification is just like protein building, a process that’s predictable and leaves few surprises.
But Ralph contends that there are a wider number of building blocks the plant has at its disposal for assembling lignified cell walls. And the plant can put these components together in a virtually infinite number of ways, like the pine trees—and many other transgenic plants—did.
This is what Ralph calls “metabolic plasticity.” As he sees it, lignification is “a remarkably evolved solution that allows plants considerable flexibility in dealing with various environmental stresses.”
Even if some don’t appreciate lignin’s evolutionary role in helping plants adapt, that’s okay, Ralph says. “A greater awareness of these plant processes will increase our opportunities to modify lignin composition and content.” Ultimately, the knowledge will lead to the development of biofuels from a third generation.
Zooming in on lignin
Another of DFRC’s many lignin-related discoveries has been especially well received in scientific circles. For the first time, Fachuang Lu, a research associate in Ralph’s group, has found a way to study the highly detailed chemical structure of the entire plant cell wall.
In the past, the job of extracting the various polymers from cell walls for detailed analysis required the deftness of a brain surgeon. There was always a tradeoff between the integrity of the material extracted and the speed with which it could be done.
Now, entire cell walls can be dissolved in a special solution in which all their contents—cellulose, hemicellulose, and lignin—are dissolved in a matter of hours instead of weeks, as with traditional methods. Once all the polymers are in the solution, NMR can provide a structural picture of them.
“Traditionally, we could only get a portion of the cell wall into solution,” Ralph says. “By using this new solution and NMR method, we can get a chemical fingerprint of the major and minor structures of the entire cell wall. The amount of detail is striking.”
Researchers interested in running cell-wall samples from either conventionally bred or genetically modified energy crops can use the tool to get a zoomed-in view of what their plants’ modified cell walls look like. With such powerful capabilities, the method can serve as an important gauge of progress.
Low-input plants for energy
In addition to probing minute cell-wall structures, DFRC scientists are also breeding plants that possess energy-friendly qualities. Casler is hanging his hopes on grasses—the perennials that cover an estimated one-third of the nation’s acreage.
Aside from switchgrass, on which he’s built an entire breeding program, Casler is also eyeing the promise of other low-input grasses, such as smooth bromegrass, orchardgrass, and reed canarygrass. He thinks they’ve got the potential to feed both cows and the country’s enormous energy appetite. An ARS researcher in Tifton, Georgia, is also looking at several alternatives to switchgrass.
Casler and colleague Hans Jung, a DFRC dairy scientist based at St. Paul, Minnesota, have been selecting grasses that possess either less lignin or fewer ferulates. “Ferulates” are chemicals that help bind lignin to hemicellulose in the cell wall, impeding access to the sugars.
“When we started these studies,” Casler says, “we wondered: Is it lignin that’s most responsible for binding up the carbohydrates, or is it the way ferulates link the lignin to hemicellulose?”
After running studies in several grass species, Casler, Jung, and collaborators have proved that either approach works when it comes to breaking down tough cell walls. Hoping to breed plants whose cell walls are more easily degraded, Casler and Jung will soon begin crossing promising grass lines.
Focusing on Alfalfa
Other DFRC researchers are focused on alfalfa—a crop that, unlike corn and other grasses, fixes its own nitrogen and so requires less fertilizer. Plant physiologist Ronald Hatfield and molecular geneticist Michael Sullivan are working to boost alfalfa’s biomass by altering genes that affect its development.
“We’re looking at alfalfa’s developmental structure, how it branches,” says Hatfield. “We’re also trying to reduce leaf abscission, or leaf drop.”
Because alfalfa plants are grown close together, many of their understory leaves fall off from lack of sunlight. Hatfield and Sullivan would like to minimize loss of this valuable plant material.
Hatfield, Sullivan, and Ralph are collaborating with the Noble Foundation in Ardmore, Oklahoma, to build the ideal alfalfa plant.
“The Noble Foundation usually engineers the plants with reduced lignin,” Hatfield explains. “Then we use NMR and other analytical techniques to see what the modified cell walls look like and how easily they can be processed either by the cow or for biomass conversion to energy.”
The alfalfa research team has already discovered that when they transform plants by down-regulating enzymes called “methyl transferases,” they can reduce lignin content, boost cellulose content, and enhance cell-wall digestibility.
Bioenergy’s just part of a bigger picture
In the end, DFRC researchers believe that agriculture’s role in supplying renewable energy to the country is crucial. But Hatfield cautions that the bioenergy movement mustn’t miss the forest for the trees.
“We need to consider the whole agricultural picture,” he says. “You can’t convert everything into bioenergy.” There are other biobased products and niche industries to consider.
Take alfalfa, for instance. DFRC researchers have found that, in addition to providing great grist for the ethanol mill, alfalfa is a source of quality protein and health-promoting nutraceuticals. Plus, its fiber fractions have value as a water-filtering agent, and it’s an ideal substrate for making an all-natural glue. (See "New Bioadhesive's a Super Glue! sidebar" for details.)
“We’ve also got to think in terms of sustainability,” says Hatfield, “for the sake of local agricultural economies and our natural resources.”—By Erin K. Peabody, Agricultural Research Service Information Staff.
Beyond switchgrass
Many researchers are investigating switchgrass as a source of bioenergy. But William Anderson, a geneticist in the Crop Genetics and Breeding Research Unit at Tifton, Georgia, says several other perennial grasses could also be developed into biofuels.
Anderson and colleagues are working with plants adapted to the southeastern United States, including bermudagrass (Cynodon dactylon), bahiagrass (Paspalum notatum), and napiergrass (Pennisetum purpureum). Each has its own advantages:
• Bermudagrass is already grown over millions of acres as forage. It is highly digestible for livestock and has good potential for conversion to ethanol.
• Bahiagrass offers less yield and lower quality than bermudagrass, but it grows well in marginal land and is easily established.
• Napiergrass, unlike the other perennial grasses, could be totally dedicated to energy use. In a 6-year study in Georgia at three locations, it outyielded bermudagrass and switchgrass by 5 tons of dry matter per acre per year. And in preliminary studies, it was converted to ethanol at a rate similar to switchgrass.
Anderson and colleagues are evaluating these grasses for desired genetic traits and breeding them for increased biomass production and better cell-wall degradability. “We’re also crossing napiergrass with pearl millet (Pennisetum glaucum), which has certain traits that reduce the amount and types of lignin, making the conversion to ethanol easier,” he says.
Image one: Structural cell wall components and third generation biofuels. Courtesy: ARS.
Image two: Chemist Fachuang Lu (pointing) and postdoc Hoon Kim view two-dimensional nuclear magnetic resonance data of a dissolved whole cell wall. Courtesy: ARS.
More information:
Agricultural Research Service: Breaking Down Walls - April 2007.
Agricultural Research Service: Probing Tiny Plant Cells to Unleash Big Bioenergy - April 3, 2007
0 Comments:
Post a Comment
Links to this post:
Create a Link
<< Home